Report: Trees for steep slopes
Dean Satchell
Sustainable Forest Solutions
dsatch@gmail.com
Reviewed by Mike Marden, July 2018.
PDF download of this report ».
Please note that the web report is regularly updated whereas the pdf download above is dated July 2018.
Plantation Forestry and Erosion
Forestry and Erosion Risk
Heavy rainfall events trigger erosion (Bloomberg et al. 2011). Duration and frequency of intense rainfall events are key factors that trigger mass-movement and gully erosion, the two most important forms of erosion in steepland plantation forests (Bloomberg et al. 2011). Slope is an important determinant for erosion risk and slopes over 32° depend on root reinforcement by trees for stability (O'Loughlin, 2005). Saturated conditions caused by heavy rainfall increases risk of slope failure and that risk becomes higher in high rainfall zones where annual rainfall exceeds 1500 mm (Bloomberg et al. 2011). Landslides "tend to initiate in slope hollows or depressions where subsurface drainage converges to produce high soil pore water pressures during heavy rainfall" (Sidle et al. as cited in O'Loughlin, 2005) and landslides are a significant contributor of sediment to water courses, even in forested settings (O'Loughlin, 2005). Shallow failures or slips tend to be a feature of steeper, concave slopes, and are usually concentrated on upper, steeper slopes (O'Loughlin, 2005).
The level with which erosion risk is mitigated from plantation forest cover depends primarily on the rotation status of the forest. For example, young plantation forests less than 6 years old sustained similar levels of landslide damage to pastured hill slopes during Cyclone Bola in the East coast of the North Island, which was 16 times greater than for plantations with closed canopies (Marden et al. 2016). This is approximately 1/4 of the total rotation length without full site occupancy.
Only once there is full root site occupancy and canopy closure do forests protect soils from erosion, therefore periods of vulnerability are influenced by two key variables – rotation length and tree growth rate (Knowles, 2006). A longer rotation length decreases the period of vulnerability (as a percentage of the rotation), provided that after clearfelling it does not take too long for newly established trees to reach the basal area sufficient to control erosion (Knowles, 2006).
Roads, landings, quarries and skid trails associated with harvesting increase the risk of erosion by disturbing and exposing soil, along with altering natural drainage patterns (Bloomberg et al. (2011). However, it should be noted that when compared with sediment contributed by gullies and landslide events, the sediment load from harvesting operations tends to be small (Bloomberg et al. 2011).
The NES-PF and Erosion Susceptibility Classification (ESC)
Since 1991, under the Resource Management Act (RMA) regional and unitary councils have been responsible for managing activities that cause erosion. However, to operate efficiently, the forest industry desired a single set of nationwide rules that regulate forestry activities with environmental outcomes, so have actively facilitated the development of a National Environmental Standard for Plantation Forests (NES-PF), which came into force on 1 May 2018.
The MPI website has a range of consultation documents and background information available for NES-PF stakeholders.
The rules and regulations
Under the NES-PF, environment regulations apply to eight plantation forestry activities that could have environmental effects in commercial plantation forests larger than 1 hectare:
- afforestation (planting new forest)
- pruning and thinning-to-waste (selective felling of trees where the felled trees remain on site)
- earthworks
- river crossings
- forestry quarrying (extraction of rock, sand, or gravel within a plantation forest or for operation of a forest on adjacent land)
- harvesting
- mechanical land preparation
- replanting.
Resource consents will be required where potential adverse effects would be significant or are not avoidable.
Erosion susceptibility classification
The NES-PF required a tool that took into account “the risks of erosion, sedimentation and environmental harm associated with plantation forestry activities in each of the classification zones” (MfE, 2011 as cited in MPI, 2017). By combining erosion severity potential identified in the New Zealand Land Resource Inventory (NZLRI) and the Land Use Capability (LUC) database, along with climatic data, a database and tool that maps classes of risk, called the Erosion Susceptibility Classification (ESC) May 2017 was produced for the NES-PF. This tool supports councils and foresters in decisions on the level of risk associated with the NES.
See the Erosion Susceptibility Classification tool here»
The ESC divides the New Zealand landscape into 4 erosion categories that are colour coded according to risk. These form the basis for determining whether a plantation forestry activity:
- is permitted, subject to certain conditions being met, or
- requires resource consent because it's on higher-risk land.
Four zones are provided in the tool that each represent different levels of risk (see Figure 1). The categories are:
- Green (low) and yellow (moderate) — land less likely to erode. Plantation forestry activities are permitted.
- Orange (high risk) or red (very high risk) — land more likely to erode. Most forestry activities can't be carried out on red-zoned land without resource consent. Some activities, such as earthworks, also require consent on orange-zoned land with steeper slopes.
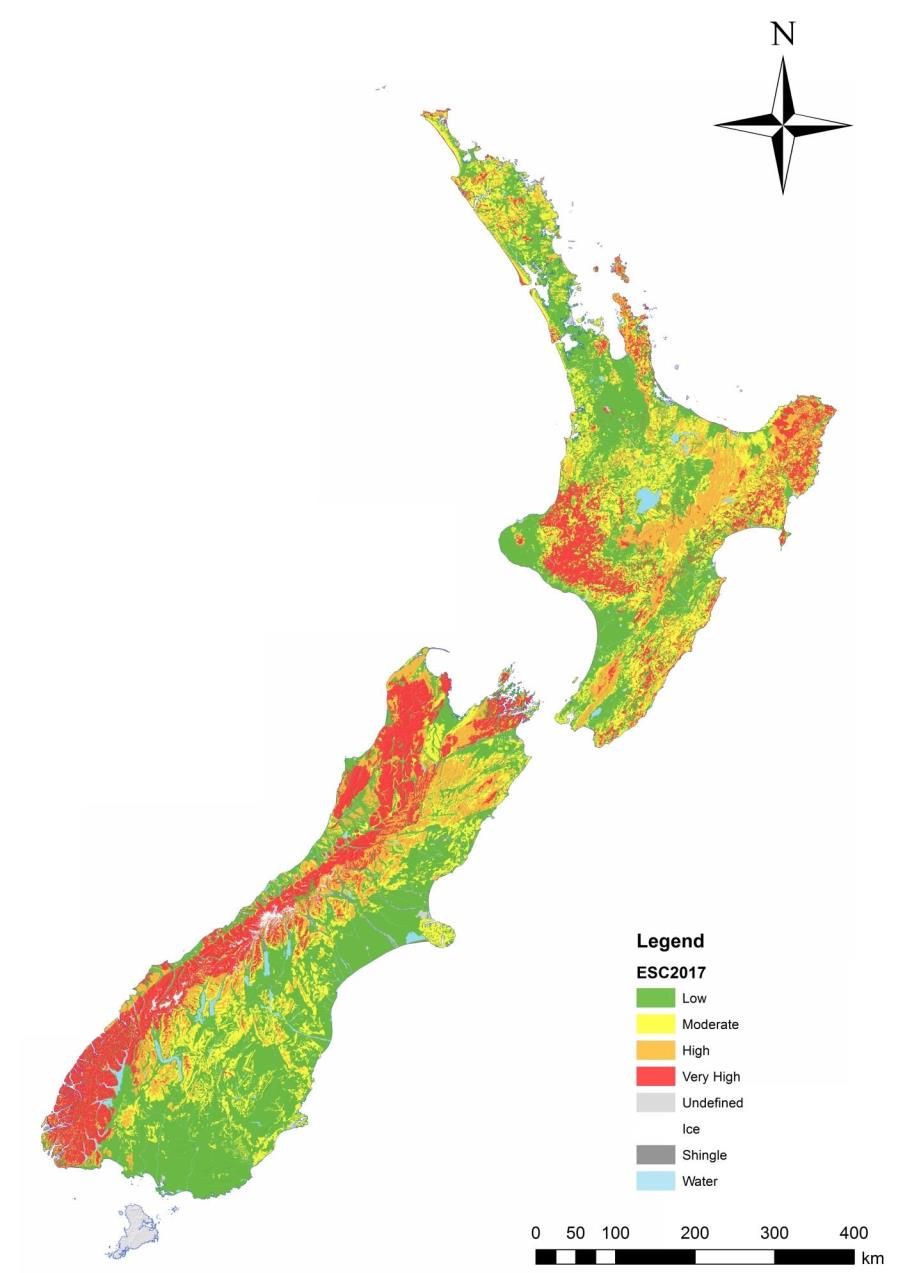
By identifying the susceptibility to erosion of the land unit in question, the ESC determines whether a plantation forestry activity is permitted, subject to certain conditions being met, or whether a resource consent is required because it's on higher-risk land. Consent may depend on conditional management practices that reduce the erosion risk above and beyond accepted levels of mitigation offered by standard forest management practices.
Potential improvements and research needs
MPI and MfE have published an overview of the NES-PF regulations stating:
For harvesting to be a permitted activity, foresters must submit a harvest plan to their local council if requested. The plan should identify environmental risks, including erosion susceptibility using the Erosion Susceptibility Classification tool, and must list the mitigations to be used to respond to those risks and achieve compliance with permitted activity conditions.
Permitted activities are subject to conditions under the regulations that are based on industry good practice standards.
Deficiencies identified with respect to the NES-PF include the need to develop an improved ESC:
- The term "erosion potential" is not well defined and is "based on subjective judgement and open to interpretation errors" (Phillips et al. 2017). Thus forest managers and territorial authorities require better tools for assessing risk at the site level. This might involve assessing the potential magnitude of erosion at the site level along with a risk assessment of likely on-site and off-site impacts and associated repair costs.
In order to better evaluate the significance of sediment mobilisation under different forest management practices, an improved understanding of the conditions that trigger debris flows and landslides is required (Phillips et al. 2017). - The scale of mapping is too coarse (1:50,000) for assessing erosion potential within a site (Phillips et al 2017).
- Probable misclassification of land (Phillips et al. 2017)
MPI have announced funding of high definition mapping of the Gisborne region to accurately assess erosion risks and better-inform erosion control decisions. This initiative intends to use LiDAR, a remote sensing tool that generates highly-accurate geographical terrain data, to assess erosion risks and assist in road design and planning. Improved management of natural hazards, such as areas of instability is a goal of this work but this would need to be undertaken at an appropriate scale in order to address inconsistencies in the ESC and improve risk assessment at the site level. Such hazard analysis is a priority for improving environmental performance and to better understand the erosion hazards associated with areas established in plantation forestry (Phillips et al. 2012).
Profiling risk as probability of landslide-causing storm events, then predicting the likelihood of failure at detailed scales and under different land management scenarios involves complex interactions between mechanical soil properties, slope, climatic factors, age of trees and individual species root network structures, that together are fraught with uncertainties (O'Loughlin, 2005). Whether this level of risk profiling for harvesting, planting and replanting on red-zoned land is necessary for the NES-PF is not clear.
Harvesting on red-zoned land (that is also LUC Class 8e) is a restricted discretionary activity where consent can be granted or declined, with consent conditions limited to the matters over which discretion is restricted. Replanting in red-zoned land after harvesting is a controlled activity, which means that consent conditions need to be reasonable and a resource consent must be granted. However, the regional council does have a level of regulatory oversight and control over the timing of the replanting and it may not be deemed appropriate to replant in exactly the same location as before, or a different species may be deemed better suited to the land slope and soil type. These decisions require judgement calls based on being well-informed on how well the proposed activity or the conditions being imposed mitigate the risk of erosion. In the short term, subjective judgement calls might be all that is available, but longer term acceptable practice might best be defined under the NES-PF.
The development of simple tools that correlate the likely frequency of landslide failure with quantitative data demonstrating the effectiveness of different erosion mitigation strategies (e.g. planting density, rotation length, species, mixed species forests with different rotation lengths etc) in reducing the erosion risk to an agreed sediment yield threshold would be useful to growers, managers and regional councils alike.
Perhaps a high level of accuracy is not required for mitigation measures that should be accepted by industry and the regulator alike as standard forestry practice for steepland plantation forestry. Conservative "best practice" erosion mitigation measures could even be described in a code of practice or standard for red-zoned land, or where necessary regulated. These practices might include:
- minimising mechanical soil disturbance when harvesting;
- high quality engineered earthworks and minimising length of roading infrastructure;
- minimising woody material left on slopes that have a high risk of failure;
- having buffers or planting setbacks between areas with a high risk of sediment mobilisation and water channels;
- ensuring rapid groundcover establishment after harvesting. This could be achieved by oversowing, immediately replanting and minimising vegetation dessication;
- replanting at a high tree stocking;
- Increasing rotation length;
- retiring excessively steep slopes inherently susceptible to landslides (e.g. > 35º) from production.
The level with which these mitigation activities should be practiced on red-zoned land could be agreed between industry and the regulator, with a track record developed that tests those practices and what they achieve over time. Measures could be fine-tuned as experience with them is improved. That is, consent for planting red-zoned land could be conditional on interim acceptance of their effectiveness at the site level.
Specific critical areas in most need of research should be addressed using forest grower levy funding. Areas identified in this report include:
- improve our knowledge of erosion mitigation provided by alternative species and regimes;
- methods to minimise slash mobilisation in debris flows from landslides;
- methods to retain debris flows so they do not reach water channels, such as tree buffers that retain landslide material;
- methods for utilising woody material to retain sediment and reduce risk of mobilisation;
- quantifying sediment retention from various types of buffers/setbacks that act as filters;
- improved establishment practices that rapidly initiate a vegetative cover after harvesting, noting that "establishment practices such as weed spraying, affect the generation, delivery, and ultimately the sediment leaving the forest." (Phillips et al. 2017);
- forest management practices that consider species and rotation length and quantify the benefits these offer in terms of erosion mitigation on steepland hill country;
- Options for transitioning unprofitable red-zoned areas to a permanent indigenous forest cover such as planted manuka, including an assessment of the duration of the window of vulnerability, potential long-term erosion mitigation effectiveness, and risk reduction as a comparison with radiata pine.
Forest harvesting and erosion
The forest canopy intercepts rainfall, reducing the quantity of water in peak flows, especially in small to medium intensity rainfall events (Fahey and Payne, 2017). Clearfell harvesting effectively eliminates evapo-transpiration, often resulting in saturated soils and increased subsurface water flow, thus increasing the risk of slip erosion (Elliot et al. 1999).
Loss of canopy from harvesting also exposes soil to direct raindrop impact and fluvial erosion (Jones et al. 2008). Tree removal allows soil to become wetter for longer (Pearce et al. 1987) and complete canopy removal by clearfell harvesting increases the risk of surface erosion and landsliding (Elliot et al. 1999; Marden et al. 2007).
Landsliding can generate large quantities of sediment and debris as a result of severe rainfall events and deliver this into streams, even in forested settings (Marden and Rowan, 1997; O'Loughlin, 2005). Shallow landslides can transform into debris flows and deliver large quantities of sediment and woody debris further down the catchment. Debris flows can also erode ephemeral gullies and riparian margins (Phillips et al. 2017).
Sheet and rill erosion resulting from earthworks, harvesting and land preparation can result in loss of soil and generation of sediment (Bloomberg et al. 2011). Although a relatively minor contributor of sediment into water channels compared with gullying and landsliding (Marden and Rowan, 1998; Marden et al. 2007), surface erosion or "slopewash" does tend to relocate sediment down-slope and can still be an important source of sediment delivery to streams, especially during severe storm events after harvesting (Marden and Rowan, 1997; Marden et al. 2002; Marden et al. 2007).
Logging systems that produce higher levels of soil disturbance and compaction produce higher levels of surface erosion, particularly in the first year after harvesting (Phillips et al. 2017). Sediment yields resulting from harvesting operations can be five times greater than pre-harvest rates, but do decline to pre-harvest levels within a few years after replant (Fahey et al. 2003; Phillips et al. 2017).
Under the NES-PF, sediment originating from harvesting must be managed to ensure that, after reasonable mixing, it does not give rise to any of the following effects in receiving waters:
- A conspicuous change in colour or visual clarity; or
- Rendering fresh water unsuitable for consumption by farm animals; or
- Significant adverse effect on aquatic life.
Soil disturbance
Surface soil horizons have the highest concentration of nutrients and greatest water-holding capacity, therefore surface soil disturbances generally reduce longer-term productivity (Elliot et al. 1999; Jurgensen et al, 1997). Under the NES-PF, stabilisation and containment of disturbed soil is required to minimise sediment entering water where it may result in certain adverse effects.
Soil disturbances are caused by wheeled and tracked harvesting machinery, by earthworks and by dragging logs across slopes. Mechanical disturbances to the groundcover remove organic material and soil litter to expose underlying mineral soil. This results in a substantial increase in slopewash generated sediment and sheet erosion (Elliot et al. 1999; Marden et al. 2006; Marden et al. 2007).
Level of sediment generation from slopewash tends to be proportional to area of soil disturbance generated from harvesting operations (Marden and Rowan, 1997; Marden et al. 2002; Marden et al. 2007). Sediment is mostly transferred from upper slopes to lower slopes, but mechanical site disturbance in proximity to streams greatly increases delivery of sediment to them (Marden et al. 2006). Where soil disturbances resulting from harvesting operations are deep, they can cause channelised flow that contributes sediment load to water courses (Marden et al. 2007; Bloomberg et al 2011).
Slopewash can be mitigated by measures such as:
- minimising soil disturbance and compaction;
- oversowing to accelerate repair of disturbances with vegetation cover that reduces the energy of the surface flow and binds soil particles in place;
- providing a riparian vegetation buffer that filters sediment (Marden et al. 2007).
Ground-based harvesting
Tracks required for skidder extraction result in substantial increases in sediment yields (Phillips et al. 2017). Ground-based clear felling operations also result in high levels of soil compaction that increase levels of fluvial erosion because soil porosity and drainage is reduced, especially when soils become saturated (Elliot et al. 1999). The area of mineral soil exposed by ground-based clear-felling operations may range from 21 – 66% (Bryan et al., 1985 as cited in Fransen 1998).
Cable hauler harvesting
Where disturbances are shallow, slopewash tends to not generate significant quantities of sediment because litter and groundcover reduces rainsplash and detachment of soil particles (Marden et al. 2006). However, deeper disturbances that expose mineral soil can comprise greater than 10% of hauler-logged cable settings (Marden and Rowan, 1997; Marden et al. 2006). These "deep" disturbances can continue to be a significant source of sediment for more than two years after logging (Marden and Rowan, 1997).
Greater depth of disturbance, especially where infiltration rates are reduced by compaction, increase sediment generation and mobility during the early post-harvesting period, especially in the first year (Marden and Rowan, 1997; Marden et al. 2007). This early period generates the most sediment because mechanical disturbance of litter exposes mineral soil to rainsplash, which detaches and mobilises soil particles (Marden et al. 2007). Without active intervention, vegetation tends to establish too slowly to reduce sediment production because newly colonising vegetation is undermined by raindrop impact and the exposed mineral soil has reduced moisture holding capacity (Marden and Rowan, 1997). Sediment generation can be greatest in the first three months after harvesting and during the first year can exceed that generated from harvest roads (Marden and Rowan, 1997). However, within one year sediment yields are significantly reduced as vegetation establishes (Marden and Rowan, 1997). After two years, in larger areas where exposed mineral soil is more deeply disturbed, vegetation recovery may only be partial, therefore they remain vulnerable to heavy rainfall events for longer (Marden and Rowan, 1997). However, the early re-colonisation process can be actively accelerated by oversowing (Marden et al. 2002; Marden et al. 2007).
Cable logging systems create fewer ruts than ground-based harvesting, covering less than 1% of the cutover area (Fransen et al. 1998). However, dragging stems across sideslopes does cause scarification of soil surfaces and haul paths from cable logging can result in soil disturbances that later form ruts (Marden and Rowan, 1997; Fransen et al. 1998; Marden et al. 2007). Sheet flow from soil disturbances can converge into rills that develop and enlarge during storm rainfall on freshly harvested ground to become ruts (Fransen et al. 1998; Marden et al. 2006). Although ruts can channel surface water runoff and convey soil material down the slope (Fransen et al. 1998), where these are generated from shallow disturbances, sediment tends to settle in micro-topographical hollows further down the slope rather than entering waterways (Bloomberg et al. 2011). Conversely, sediment generated within deeply scoured haul paths located in close proximity to stream channels generally enters waterways (Marden and Rowan, 1997).
The extent of deep soil disturbances caused by hauler logging can be reduced by:
- ensuring adequate log lift over as much of the haul length as possible;
- uphill-hauling logs away from stream channels rather than across them; and
- harvesting smaller areas so that fewer logs cross the same ground (Marden et al. 2007).
The NES-PF requires butt suspension ("suspending the sawn base of the tree being harvested above the ground or surface of a water body while pulling it to a landing”) wherever practicable. However, sufficient lift to keep logs off the ground is not always possible in some terrains. There is a trade-off between building more roads, tracks and landings that generate sediment, and greater levels of soil disturbance resulting from hauling in less than optimal conditions, where the logs get dragged across slopes (Marden et al. 2002).
Because deep scouring is rare in well-managed hauler logging operations (Fransen et al. 1998), harvesting with cable haulers may be the only option for steepland with fragile soils (Raymond, 2012). However, the cost of extraction is generally at least double that of mechanised ground-based systems (Raymond, 2012), an important consideration given that the proportion of forest best suited to hauler logging (i.e. slopes greater than 20 degrees) was 44% of the total area harvested in 2014, and is forecast to rise to 53% by 2016, and to over 60% by 2025. (Moore, H. 2014).
Earthworks
Forested catchments may yield up to 80% less sediment than pastured catchments (Phillips et al 2017). However, earthworks can generate large amounts of sediment by causing mass movement or surface erosion (Basher et al. 2016b). Such erosion-generated sediment can be controlled very effectively by "hard" engineering works that prevent sediment from being discharged off-site, or that prevent erosion from occurring by diverting water flow. Susceptibility to erosion from earthworks can be minimised by carefully designing drainage channels and roads to an engineered standard (Bloomberg et al. 2011). Engineering works can:
- reduce water velocity to manage runoff;
- modify slope hydrology to reduce mass movement erosion;
- trap sediment before it moves into waterways; and
- improve streambank strength to resist hydraulic scour (Basher et al. 2016b).
Export of sediment may be prevented through appropriate structures such as dams, silt traps or wetlands (McIvor, 2011) and careful siting of roads and landings (Basher et al. 2016b).
The forest industry has become very active in managing the environmental effects of earthworks by careful planning that ensures best-practice engineering design and construction practices. These practices have significantly reduced sediment yields from landing and road construction earthworks (Phillips et al. 2012). The Forest Owners Association have published the Code of Practice for Plantation Forestry (2007) and the Road Engineering Manual (2012), as guides to best management practice. However the contribution that infrastructure and clear cut forestry operations have on generation of sediment is not yet well characterised (Basher et al. 2016b).
Roading
Because roads inherently have low hydraulic conductivities, surface runoff creates a high risk of causing soil erosion if this is not managed (Elliot et al. 1999). Harvest roads and tracks are a major source of sediment supply to streams (O'Loughlin, 2005). That said, surface erosion of roads by fluvial action produces significantly less sediment yields than mass movement erosion, where this occurs (Bloomberg et al. 2011).
Sidecutting of steep slopes for roading decreases site stability - the cut side is likely to slip and the fill side may be too steep and fail as slumps, landslides or even avalanches (Bloomberg et al. 2011).
Harvest systems that require higher levels of road, track and landing construction generate greater levels of slope disturbance and inherently increase the potential for slope instability (Phillips et al. 2012).
Location of roads should recognise erosion hazard, such as avoiding unstable ground and locating roads on ridge tops terraces and benches (O'Loughlin, 2005) and use appropriate construction methods to prevent landslides (Phillips et al. 2012), including "catering for drainage, avoiding extensive side casting on steep slopes and vegetating new cut and fill slopes" (O'Loughlin, 2005).
As road age increases, the risk of erosion increases (Bloomberg et al. 2011). Therefore road maintenance should be undertaken at regular intervals to prevent high costs associated with remedial works.
Limiting harvest operations to periods of dry weather may allow harvest machinery access to a plantation area where roading standards are limited by cost, while also managing erosion risk (Palmer, 2013a).
Woody debris
Wood is an integral part of waterway ecosystems where it is transported down through the system, gradually breaking down from abrasion and decay, with some woody debris ending up in lakes, estuaries and coastlines (Ballie, 2005). Large woody debris, if well anchored in waterways, have a significant positive impact on the stream ecosystem. This impact is greatest in smaller waterways with lower flows because woody debris are less likely to be dislodged (Ballie, 2005). Anchored wood (Ballie, 2005):
- slows down water flow thus dissipating energy that could otherwise cause scouring and carry sediment downstream;
- helps aerate water and increase residence time, thus improving microbial decomposition of organic material;
- provides food resources and habitat for fauna.
However, because most of the larger pieces are removed at harvest, smaller logs and pieces that end up in the stream channel may not anchor well. Being so mobile, during high water flows this material may form debris flows and cause damage downstream, so removal of these from streams is common practice (Ballie, 2005).
Mobile sediment can be constrained by the presence of harvest debris (stem and branch material) by slowing down the velocity of water flowing overland (Marden and Rowan, 1997) so these have potential as a tool for actively mitigating post-harvest soil losses from entering streams. This could include positioning larger structural material to hold smaller material and sediment in place where sheet erosion occurs and in ephemeral channels and smaller more stable waterways (Ballie, 2005). Such active management would need to ensure that woody debris do not become mobilised and delivered into larger water channels during a severe storm event. Slash traps are a permitted activity under the NES-PF for the purpose of catching larger pieces of slash that would otherwise be flushed out of the catchment in high flow conditions (MPI, 2018). Design must allow water to flow freely through the slash trap, which must be maintained and reported on to the Regional Council annually, detailing frequency of maintenance and clearance along with condition and performance. This is to ensure large quantities of slash do not build up and become mobilised during heavy rainfall events (MPI, 2018).
Under the NES-PF slash and debris must be managed appropriately:
- Slash from harvesting must be placed onto stable ground;
- Slash from harvesting that is on the edge of landing sites must be managed to avoid the collapse of slash piles;
- Slash from harvesting must not be deposited into a water body or onto land with a high risk of flooding.
Debris flows
Landslide-generated sediment when mixed with woody debris can form a debris flow and if confined to a channel they become far more destructive than water alone (Scion, 2017).
Where slopes are steep and susceptible to mass failure, the risk of debris flows is increased (Scion, 2017). Debris flows are most likely to occur during intense rainfall events in the post-harvesting "window of vulnerability" period (Scion, 2017). Where there is slope failure with larger slash and log residues present, the destructiveness of debris flows increases, with negative consequences downstream as unwanted debris dams or the accumulation of material along coastlines or in lakes (Bloomberg et al. 2011). Debris flows also erode the water channel that carries them, so can carry large amounts of sediment downstream (Scion, 2017). Deposition of sediment then aggrades water channels, increasing the risk and severity of flooding events (Marden and Rowan, 1997).
Managing the occurrence of debris flows and minimising their impacts involves reducing the risk of landslide occurrence, by
- reducing the duration of the window of vulnerability after harvesting; and
- extending the rotation length (Scion, 2018).
Vegetation management
Vegetative cover enriches and protects soils from erosion. A strong regeneration of weeds after harvesting was found to be important in mitigating erosion (Fransen, 1998). Conversely, the desiccation of weeds that compete with or prevent rapid establishment of tree seedlings, or the application of residual herbicides that prevent vegetation from establishing, may facilitate surface erosion processes during storm events (Bloomberg et al. 2011). Because plant roots bind soil particles at the soil surface, rapidly reinstating a vegetative cover controls surface erosion and avoids undercutting and increases slope stability (Phillips et al 2012). The sooner vegetation cover recovers after harvest (in contrast to soil remaining fallow), the less soil is lost as surface erosion, especially from ruts and soil disturbances in the logged setting (Fransen, 1998). Undercutting of slopes by fluvial erosion in heavy rainfall events can even trigger landsliding (Phillips et al. 2012).
Weed control in radiata pine forests generally involves releasing trees by applying herbicides two or three times in the first few years (Ronaldo and Harnett, 2015). Although this practice of releasing young radiata pine from weed competition is well known to accelerate tree growth, weed competition can also have a beneficial effect on tree form, with fewer multiple leaders and malformations, less branchiness along with less toppling and sweep (Mead and Lucas, 2008). There may be an opportunity for steepland forest managers to explore the tradeoff between encouraging vegetation to establish rapidly on a recently harvested site to mitigate erosion, and limiting establishment of competing vegetation on the site for ease of tree establishment and early tree growth rates.
Forest Management and Erosion
"In the 1970s we anticipated that appropriate harvesting techniques for gully planting would be in place before harvest for small radiata plantings, but regretfully this has not happened. Until it does we need fast-establishing and slow-growing trees with quality wood which can be harvested in small lengths for niche markets" (May, 2017).
Erosion reduces forest productivity by reducing soil water availability, removing plant-available nutrients and degrading soil structure (Elliot et al. 1999). A range of harvest management options are available to assist forest managers in mitigating the risk of erosion. For example harvesting options include clearfelling (high risk), or single tree harvesting (low risk) thereby maintaining a permanent canopy cover.
Scale of the operation, the cost of access and the cost of harvesting are important considerations for managing steepland forest plantations, especially where the species is selected for production of lower value commodities (e.g. radiata pine). The steeper the terrain, the more difficult access becomes and the further from the port the less profitable the forest will become (Moore, 2015). Managing erosion may result in steeplands being under permanent forest cover and a change in species to one with a longer rotation and a higher timber value, or where uneconomic retiring the land from production with reversion to natural forest cover.
Also, the risk of windthrow is an important consideration in the selection of management and harvesting regimes. The likelihood of windthrow losses increases immediately following thinning (Somerville, 1980; Somerville, 1989), especially in clearfell systems with the goal of maximising volume and diameter within a time frame, whereby thinning may result in widely spaced trees with a high height to diameter ratio (Perry et al. 2015). Conversely, irregular forest structures such as those under a continuous cover, tend to have lower height to diameter ratios which could increase wind-firmness (Mason, 2002; Schelhaas, 2008).
Scale of operation
Reducing the cost of harvesting can significantly improve profitability and harvest production efficiency improves as harvest area increases (Raymond, 2012). Reducing cable logging costs for smaller harvest volumes is challenging (Raymond, 2012). Given that small-scale forests are less efficient to harvest, Moore (2015) suggested that one solution to improve cost-efficiency is for "small-scale forest owners to work collectively to maximise the profitability of these forests". However, where retrievable volumes are low, machinery costs will be high regardless of the size of the area being harvested.
Efforts in New Zealand to improve the efficiency of cable operations while also eliminating manual chainsaw felling have resulted in the successful use of excavators to fell and bunch logs on slopes (Raymond, 2012). Delimbing in the forest reduces stem weight, enabling extraction with smaller faster cable yarders, which "has led to increased adoption of mechanised harvesting on steep land, taking advantage of improved production, lower costs and improved safety" (Raymond, 2014).
Feller buncher machines have been developed in New Zealand that are capable of working on slopes in excess of 45 degrees. However, the large machinery as used in high production harvesting (i.e. bunching and grapple extraction) is not cost effective for small-scale harvesting operations, particularly where sites are scattered, because of the costs associated with relocating heavy machinery (Raymond, 2014).
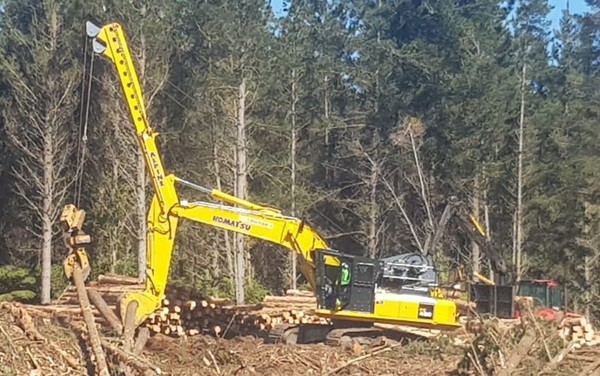
Lightweight yarders with an operating weight of 45 tonnes and designed to work at a high production level can cost-effectively harvest smaller and steeper forests (e.g. Log Champ LC 550) and the excavator-based Harvestline yarder offers efficient log extraction in difficult, short to medium distance cable extraction areas, with a maximum effective haul distance of 350 m. However this requires expensive and heavy machinery (a 35 tonne plus excavator) which inevitably increases the harvesting costs, but has minimal environmental impact.
Skidder-mounted cable haulers with log extraction to a roadside log storage yard is another practical option for smaller-scale harvesting that avoids ground-based systems and can produce significant economic returns on challenging steep sites (Palmer, 2013a). Other options for small-scale steep slope harvesting include modern small-scale cable logging (skyline) systems such as the Maxwald mini skyline system (ForInco, 2016). The Maxwald mini skyline system requires greater than 17 degrees slope, has a maximum load of one tonne, and requires a extractable volumes of 30 m3 per corridor (300 m maximum extraction distance) to be economically viable (ForInco, 2016).
Continuous cover forestry
Under the NES-PF harvesting is a permitted activity on red-zone land provided a 75% canopy cover is maintained at all times (low intensity harvesting).
Silvicultural systems that maintain the forest canopy and mimic natural successional processes are considered to be a more sustainable forest management practice than clearfell harvesting (Barton, 2005). Maintaining the complete forest cover offers improved soil and water values (Barton, 2005). Continuous cover forestry encompasses a range of practices from small coupe harvest removals to single tree extraction (Barton, 2005). In the United States clear-cut harvesting practices are being replaced by partial-cut harvesting under ecosystem management principles in an attempt to manage erosion (Elliot et al. 1999). However, limiting the size of clearfell coups presents both operational and economic challenges (Bloomberg et al. 2011).
Managing forests under continuous cover requires an intimate understanding of the site requirements of each species being considered (Barton, 2005). For example species requiring high light levels should be harvested in larger coupes. Larger coupes are considered to be no wider than two tree heights, thus limiting coupes to no more than a quarter of a hectare in area (Barton, 2005).
Although the approach is similar to the planting of an even-aged forest in a single species, greater attention is required in the siting of each species by understanding its ecological requirements, while also considering site soil quality and climatic conditions (Barton, 2006). For example exposed sites may require nurse crops or high initial stocking, fertiliser may be required for poorer soils, species must be selected according to soil moisture availability and unwanted regenerating species controlled (Barton, 2006). Forward planning might predetermine coupe size, stocking and rotation length for the forest to produce a continuous supply of logs of the desired volume and size range (Barton, 2006).
Forest improvement practices focus on individual trees and those stems with the best quality wood. Interventions that optimise returns consider individual tree regeneration and increment rather than clearfell considerations such as age and stand volume (Barton, 2005). This involves actively managing the forest for optimum productivity, with interventions such as timely thinning of poor quality trees and the removal of mature crop trees to ensure that the remaining trees have sufficient room to grow (Barton, 2006). Maintaining the vertical structure of the forest should aim for greater structural diversity, with "tiers" that improve longer-term productivity by allowing dominant trees to put on as much volume as possible, while also ensuring that subdominant trees are positioned to put on growth once the dominant trees have been removed (Barton, 2006).
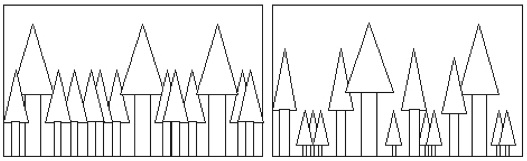
Maximum stand density index (or basal area) for an even-aged stand requires that the target tree size increases as stocking decreases. That is, as target diameter increases, fewer trees can be grown in an even-age stand, therefore less use is being made of available growing space for volume production during the growth period. In contrast, multi-age management opens opportunities to harvest large target diameters and also maintain a high level of site occupancy, by increasing the frequency of harvesting operations and harvesting fewer trees each time (Berrill and O'Hara, 2007). Continuous cover methods practiced in California redwood stands involve predetermining the harvest cycle (time period between harvest operations) and setting target diameter, which then dictates number of trees harvested in each cycle (Berrill and O'Hara, 2007). With a single cutting cycle (i.e. two-aged stand, Figure 2), basal area may be reduced by up to 80% in a single harvesting operation (Berrill and O'Hara, 2007). Averaged over time, by increasing the frequency of cutting cycles, a greater forest cover is achieved (Berrill and O'Hara, 2007). Productivity is maximised with individual cycle lengths as short as possible, while profitability is a tradeoff between higher harvesting costs for shorter harvesting cycles and maximising volumes harvested of high quality large diameter trees (Barton, 2006). Managing individual cycle lengths to be as short as possible also carries with it a greater risk of harvesting damage to the residual stand (Berrill and O'Hara, 2007), so management requires multiple trade-offs.
Maintaining records of the distribution of trees within size classes allows actual and desired proportions for each size class to be compared, with the objective of optimising stocking and maximising growth increment. Harvesting of trees is limited to those that have achieved the target diameter, thereby ensuring annual harvest volumes are maintained at or below the annual incremental increase in stem diameter (Barton, 2006).
Continuous cover forestry is more likely to be economically viable if the timber is of sufficiently high value to offset harvesting costs (Wallwork and Rapley, 2009). On steeper country where roading is impractical, helicopter extraction is an option that ensures minimal damage to soil, but to be economically viable this also requires timber values to be high (Barton, 2005). Although high value timber trees may remain economically viable despite the high cost of using more sensitive harvesting systems (May, 2017), it is worthwhile noting that “marketing of small quantities of logs is always a problem” (Van Kraayenoord and Hathaway, 1986).
Following harvesting, management practices should aim to encourage the growth of the residual stand. This may involve removing weeds and reducing tree stocking to an optimal stand density. Regular releasing should also be practiced (Barton, 2006).
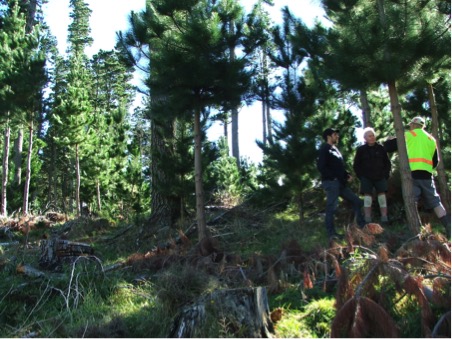
Farm forester John Wardle practices continuous cover radiata plantation forestry near Oxford, Canterbury, using a single tree selection system called target diameter harvesting (TDH). Single trees are harvested with the target diameter (DBH) being 60 cm, with consideration also given to tree form, gap creation and spacing of residual trees (Sharland, 2008). However, "Very precise controlled directional felling is essential to prevent damage to the residual crop and to protect existing regeneration" (Sharland, 2008). Trimming, log making and cross cutting all take place at the stump, with logs skiddered to a mini-landing before being transported off site (Sharland, 2008). An economic evaluation of Wardles "Target Diameter Harvesting" (TDH) system concluded that similar returns to those achieved for clearfell harvesting were possible, but with improved ecosystem services (Perry et al. 2015). Harvesting only 15 trees/ha/yr-1 produces 40-50 m3 of logs, with returns of $1300/ha/yr-1 in perpetuity, with thinning getting a growth response even in large trees (J. Wardle, pers. comm). Through a pioneering process of adaptive management Wardle is increasing tree densities per hectare and carrying larger standing volumes (J. Wardle, pers. comm).
A project is currently assessing the practical requirements and constraints of introducing the TDH system to forests of different sizes, along with the economic feasibility of such systems (NZFFA, 2018).
Spaced tree plantings
Space-planted trees are a widespread method used by hill-country pastoral farmers to control erosion. Tree species planted are usually poplars and willows, but other species such as Eucalyptus and Acacia are occasionally used (Basher et al, 2016b). However, no studies have measured their effectiveness under differing severity of storm events, nor on sediment yield (Douglas et al. 2009, as cited in Basher et al. 2016a).
The success of space-planted trees in controlling landslide erosion depends both on good initial establishment of the trees, along with subsequent maintenance to ensure good root and canopy development (Basher et al. 2016a). Increased tree size and planting density both reduce risk for landslide erosion to occur, but there has been limited work quantifying these relationships (Basher et al. 2016a). These limited studies suggest that only once mature, do trees planted at wide spacings significantly reduce landsliding, and then only within a distance of 10 m from the trees. Younger and smaller space-planted trees offer little protection and are only effective once diameters reach 20-30 cm (Basher et al. 2016b). The interaction between roots of neighbouring trees is a major factor determining success of close tree spacing in preventing erosion and therefore levels of erosion reduction attained by space planted trees are nowhere near the level attained by forest cover (Basher et al. 2016a). Although a clear improvement over having no trees at all, sparse tree cover and a long window of vulnerability that is dependent on tree spacing and size offer limited mitigation benefits.
Agroforestry and tree stocking
In converting pastoral land to forestry, grazing can be retained between trees for a period of time. By decreasing initial tree stockings and retaining lower residual stockings, pastoral productivity is retained for longer. With a low final crop stocking of 200 stems per hectare, grazing is available for half the rotation, but productivity is halved during that time (Mead and Lucas, 2008). Agroforestry (i.e. low tree stockings) on steep slopes is therefore a trade-off between risk of erosion, pastoral productivity and poor wood quality resulting from heavily-branched trees that also reduce returns because of high harvesting costs.
Toppling is a considerable risk for low-stocked stands in that gaps in the canopy both increase erosion risk and also reduce productivity. Physiologically aged cuttings can result in lower levels of toppling compared with seedlings (Mead and Lucas, 2008; Moore, J. 2014).
On fertile pastoral sites a low tree stocking to retain grazing results in large logs and a shortened rotation (Hawke, 2011). However, the trade-off for the fast diameter growth rates produced by individual trees under low stocked regimes is a low total harvest volume and with low value branchy headlogs (Hawke, 2011), which can produce poor returns (Jones and Cullen, 2008). Mason (2007) and Hawke (2011) explained the trade-off between volume and value, whereby low stocking rates increase volume of pruned buttlogs at the expense of total volume. Hawke (2011) found that top breakages and poor stem straightness were both prevalent in low-stocked stands, whereas stands with higher tree stockings were largely unaffected. Seymour (2017), on the other hand, produced good returns from 25 year old hill country radiata planted at a stocking of 150 stems per hectare, by using improved cutting grown stock and high pruning to 8.5 metres in five regular and well-timed lifts, to retain a small DOS and maximise clearwood recovery and buttlog volume. Nevertheless, larger trees with heavier branches do challenge the cost-efficiency of mechanised harvesting systems (Raymond, 2012).
Agroforestry at low tree stockings, especially where soil conditions are good, produces fast diameter growth that results in large branches and also poor wood stiffness, limiting use for structural framing applications (Bawden, 2007). In contrast, full site occupancy produces slower diameter growth (closer growth rings) and greater wood stiffness (Bawden, 2007; Hawke, 2011). Mason (2007) also found that wood stiffness improves by increasing between-tree competition. Therefore, production of better structural timber grades from headlogs, along with large diameter pruned buttlogs, while also mitigating erosion risk on steep slopes, would require high initial stockings to minimise the volume of juvenile wood, with a progressive series of thinnings each time full site occupancy is achieved.
Bawden (2007) also suggested that because steeper slopes tend to have less soil moisture and nutrients available to trees, wood properties such as density and stiffness are improved because of the resulting slower growth and longer rotation length. Longer rotations also reduce erosion risk and once age exceeds 30 years, radiata wood tends to have improved stiffness properties (Bawden, 2007). Longer rotations are also preferred by log buyers but do come at an economic cost (Mason, 2007).
As tree stocking increases, a greater volume of wood is produced per hectare (Hawke, 2011). With no thinning and at planted spacings of 6 m x 6 m good returns were reported for high pruned radiata harvested at 26 years old, with 78% of net returns coming from pruned logs (Mackenzie, 2017). Moore and Harnett (2016) reported that a final crop stocking of between 400 and 600 stems achieves the greatest standing volume per hectare and that stocking rate is the key determinant for value.
Prices for different log grades also tends to influence management decisions on tree stocking and silviculture. Improved seedlots produce trees with better branch architecture and improved stem straightness, and therefore improved log grades and greater returns (Moore and Harnett, 2016). However, as seedlots are improved for growth and form, deploying these at lower stockings has the potential for increasing erosion risk on steep hill country.
Managing post-harvest landslide risk
Although tree roots reinforce the soil and prevent erosion, the tree canopy also makes the soil drier which increases soil strength (Marden et al. 2016). When the trees are removed, the soil is directly exposed to rain and therefore surface erosion, but also to increased water infiltration, which increases risk of landslides occurrence. Then, as tree roots decay, their soil reinforcement capacity is lost. Although mature plantation forests control erosion very well, there is a period of between two and eight years following harvest where slopes are vulnerable to erosion (Phillips et al. 2012 as cited in Basher et al. 2016b). This is known as the "window of vulnerability", which has a significant influence on the risk and magnitude of damage caused by storm-induced landslides (Marden et al. 2016).
Until trees achieve full root occupancy and canopy closure occurs, erodible slopes remain vulnerable to landslides (Marden et al. 2016). Radiata pine plantations less than 6 years old and planted at moderate stockings do not have sufficient site occupancy to reduce erosion (Marden, 2004).
In addition to rotation length, harvesting methods and silvicultural practices (in particular planting tree density and tree growth rate) also influence the risk profile for post-harvest erosion to occur (Phillips et al. 2015a).
Harvest risk and the window of vulnerability
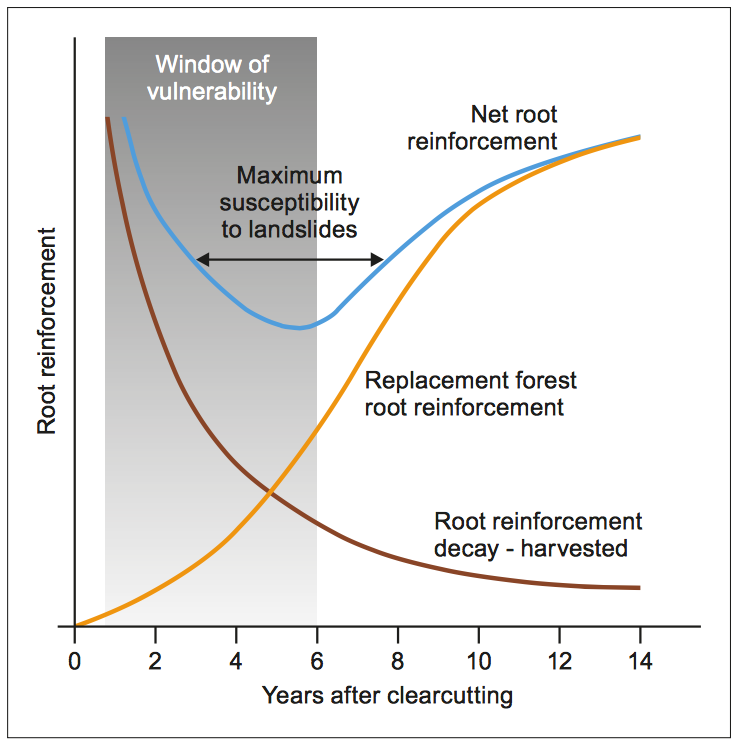
The period of maximum susceptibility to mass movement is between 2-8 years after clearfell harvesting (O’Loughlin, Sidle, Watson as cited in Phillips et al. 2012). See figure 3.
The risk of sheet erosion and rill erosion is greatest immediately after harvesting because of soil disturbance and removal of forest cover.
Rotation status and level of canopy closure both influence the level with which erosion risk is mitigated: Factors that influence this include:
- A forest canopy intercepts rainfall and increases evaporation of moisture, resulting in lower soil water balances (Bloomberg et al., 2011; Basher et al. 2016b; Pearce et al. 1987).
- Tree root growth and resulting level of root reinforcement increases with age from planting. This is more important than canopy interception in terms of mitigating erosion (Sidle and Ochai 2006).
- Forest litter accumulation has soil building effects such as improved soil aggregate stability (Bloomberg et al., 2011 p 23) and increased hydraulic conductivity (Elliot et al. 1999) that mitigate erosion risk.
As stumps and roots decay, root reinforcement of soils is reduced (Basher et al. 2016b). Rapid development of a replacement stabilising root network is important for reducing risk of mass-movement erosion events. As root site occupancy increases with age of the replanted stand after clearfell, landslide risk reduces. However, the length of time between harvest and root decay is species dependent (Phillips et al. 2012). Radiata pine roots decay quickly and lose half their tensile strength within 15 months of harvesting (Watson et al. 1999), resulting in significantly reduced soil reinforcement. The new soil-stabilising root network in the replacement crop is likely to be slower to establish than the decay-induced loss of reinforcement resulting from harvesting (see Figure 3). Clearfell harvesting of radiata pine results in a period of time where risk of erosion is substantially increased (Watson et al. 1999). Post-harvest slope stability is therefore influenced by:
- tree stocking rate
Increased planting densities offers faster development of a stabilising root network; and - tree species
A species with slower rate of root decay than radiata pine offers a longer time period before soil reinforcement is lost.
Replant stocking rate is also dependent on growth rate of the species planted because faster growth results in a shorter time period for recovery of root reinforcement (Phillips et al. 2012).
Because erosion of soil tends to reduce productivity over much longer time frames than a single rotation (Elliot et al. 1999), clearfell harvest frequency also influences the proportion of the harvest cycle that has insufficient root reinforcement prior to canopy closure (Bloomberg et al, 2011).
Species
The window of vulnerability occurs during the first 6-8 years of a typical radiata pine rotation (Phillips et al. 1990). Rate of decay for the roots of the removed trees also influences rate of root reinforcement loss (Phillips et al. 2015a). Once the stem is cut, radiata pine roots decay rapidly and lose their strength such that "within a few years little can be seen of the root system in the soil" (Watson et al. 1999 as cited in Phillips et al. 2012; see also O’Loughlin and Watson 1979).
Knowles (2006) concluded that radiata root strength has a "half life" of 15 months after harvesting, implying a progressive loss of root strength that halves again after 30 months. Kanuka roots, on the other hand, were estimated to decay at only half the rate of radiata pine roots (Watson et al. 1999). This suggests that rate of decay is strongly influenced by species.
For some species, the cut stem and root system does not die. For example redwood "retains both the physical stump that continues to act as a ‘buttress’ as well as its intact ‘live’ root system" (Phillips et al. 2012). Other genera that include coppicing species include Eucalyptus, Acacia and Populus. In terms of soil reinforcement and effectiveness at controlling erosion, because such root systems remain alive they continue to occupy the soil and reinforce it. (Phillips et al. 2012).
Root site occupancy
The number of years required after trees are planted and before protection is afforded against shallow landslides depends on species composition, spacing of trees and growth rates (Marden et al. 2016). The threshold that closes the window of vulnerability is in theory the point where roots of adjacent trees overlap, referred to as "100% root site occupancy" (Phillips et al. 2011). Canopy closure also occurs around this time (Phillips et al. 2015a). The length of time to reach that threshold is primarily determined by early growth rate of the species being planted and the planting density (Phillips et al. 2015a; Marden et al. 2016). Earliest protection is provided by full root site occupancy (root overlap) and canopy closure, i.e. species with the fastest growth rates or those planted at high densities (Marden et al. 2016; Phillips et al. 2015a).
For reliable landslide protection, tree species "that have wide environmental tolerances are also likely to be preferred over species that are less widely tolerant" (Phillips et al. 2015a). Radiata pine grows well throughout much of New Zealand and is easy to establish (Maclaren, 1993). However, wide tolerances do not necessarily mean better adaption to specific soil physical conditions such as poor drainage, low soil nutrient availability or shallow soil depth. Root system morphology is also closely associated with site factors (Phillips et al. 2015a) whereby different species adapt in different ways to such site factors. Species preferences and limitations are therefore important for successful establishment and capacity to prevent erosion.
Rate of root biomass production varies between species for individual trees of a given age (Basher et al. 2016a). For example, eight year old radiata at a stocking of 2000 stems per hectare has a similar level of landslide risk to kanuka of the same age with a stocking of 10,000 stems per hectare (Ekanayake et al. 1997). To compensate for its smaller roots at all stages of growth, kanuka requires higher stand densities (Ekanayake et al. 1997). Where stand densities are very high (between 15,000 and 25,000 trees), for the first eight years "kanuka would provide a better level of protection against the initiation of shallow landslides than stands of planted and managed P. radiata" (Ekanayake et al. 1997). Knowles (2006) also found that species with less root biomass tend to have greater tensile strength, whereby comparative soil holding ability should be similar for all species, provided above-ground biomass is equivalent. Therefore tree size and stocking may be much more important than species in terms of root reinforcement (Knowles, 2006).
Planting density/tree stocking
Because of cost constraints and an improved selection ratio of 3:1 resulting from breeding of genetically improved radiata seedlings, initial spacing for radiata pine has been reduced from 2,200 stems/ha-1 in the late 1920's to current planting stockings of between 800 stems and 1200 stems/ha-1 (Sutton, 2007).
The Ministry of Forestry (1994) recommended a planting density of 1250 stems/ha-1 on eroding land classes in the East Coast. This recommendation was based on a site occupancy model that used time-series data of crown and root diameters according to age and planting density (Marden et al. 2016). However, because root diameters were not known for radiata less than 8 years old, growth rates for this critically important establishment period in terms of vulnerability were only estimated (Marden et al. 2016). Marden et al. (2016) sought to improve the time series database for younger trees and tested genetically improved radiata to see whether faster growth rates would provide earlier root reinforcement compared with less improved radiata planting stock (such as was available from the 1960's onwards). Total root length, root cross sectional area and canopy growth rate were not significantly larger for genetically improved (GF 28) trees compared with GF 16 radiata at age four, suggesting that genetically improved planting stock may not necessarily provide an opportunity to reduce stocking without increasing risk of erosion (Marden et al. 2016).
Quantifying root reinforcement provided by trees involves the interaction between tree size and tree spacing (Schwarz et al. 2016). Tree size is influenced by growth rates for different species, which in turn is influenced by site factors (Knowles, 2006). As trees grow, radiata pine produces on average 1.1 tonnes per hectare of root biomass per annum for the first 9 years of growth, which increases to 7-8 tonnes per hectare by age sixteen and 9-10 tonnes per hectare between sixteen and twenty-five years old (Watson and O'Loughlin, 1990). Risk of erosion decreases as tree biomass production increases (Ekanyake et al. 1997).
Knowles (2006) found a strong relationship between basal area and root reinforcement (soil holding ability), with "a minimum threshold of 30 tonnes of radiata pine root biomass per hectare". Thus tree stocking and tree size when combined as basal area represent total biomass (for example 12,000 trees at 5cm dbh or 90 stems at 45 cm dbh both produce Knowles's 30 tonnes per hectare risk threshold). Canopy closure for typical radiata occurs between 6 and 8 years after planting, at which time the root systems are sufficiently well developed to reinforce the soil and minimise risk of landsliding (Marden et al. 2016). However, Knowles's model may require refining to more accurately profile a risk basal area threshold during the key establishment period when soils are most vulnerable and when thinning operations are undertaken, given that both initial tree stocking and thinning operations both affect canopy and root network areas (Bloomberg et al. 2011). Marden et al. (2016) suggested that when radiata is thinned, usually between 5 and 8 years after planting, despite widening of the canopy gaps, root development should be sufficient such that slope stability is not compromised.
What is clear from the literature is that root reinforcement occurs more rapidly at a higher stand density and increases over time. Watson et al. (1999) estimated that replanting radiata at 1250 stems/ha-1 after clearfell on erodible steep slopes results in a period of 4.7 years where root reinforcement is reduced, increasing to 5.6 years where replant stocking is reduced to 800 stems per hectare. The question is therefore: How high should stocking be, given the risk profile for the land being planted?
Historical evidence shows that densely planted stands afford protection from storms earlier than stands planted at lower stocking rates (Marden et al. 2016). However, the trend towards lower stocking rates for radiata, such as 833 stems per hectare (because of improved establishment practices and planting stock) has been found to increase the period of vulnerability and risk of erosion on slopes. Where planted 2 m apart, the canopy of adjacent trees touched within three years, whereas where planted 4 m apart this did not occur until between six and seven years of age (Marden et al. 2016). Although root spread increased significantly for each year the trees grew and by year 4 had reached a maximum of 6.04 m from the stem, at 1250 stems per hectare (4 m x 2 m) only 20% of total root length overlapped with roots of adjacent trees (Marden et al. 2016). Importantly, Marden et al. (2016) considered that radiata at this stocking and age, even with the gains in growth from genetically improved planting stock, would not provide "lateral and/or vertical root development to prevent the initiation of shallow landslides should a major storm event coincide with this early growth period".
Thus the "duration of the window of vulnerability" that culminates with the decay of the root systems of harvested trees needs to be shorter than the time it takes for the new root network to attain full root occupancy and thereby maximise net root reinforcement. Decisions on stocking rates could therefore involve a cost benefit analysis by the landowner, which would also take into account the probability of a landslide-triggering event occurring during the period of time it takes for the new crop to achieve 100% root reinforcement (Bloomberg et al. 2011). Sites with a higher risk might require a higher initial stocking than is current standard practice.
Although the level of root reinforcement required to stabilise a specific slope depends primarily on mechanical soil properties and slope (Schwarz et al. 2016), a much improved understanding of landslide thresholds is required for a range of different storm profiles in order to more accurately assess risk for each site (Phillips et al. 2015a). Risk-based regional mapping that links forest slope reinforcement models with levels of erosion susceptibility could provide such a risk profile (Phillips et al. 2015a) and aid decisions on planting stockings.
Silvicultural regimes would also need to be altered for very high initial stockings, with a higher number of thinning interventions required to progressively lower the stocking to an appropriate level for the desired rotation length. Sutton (2007) reported historical experiments with high initial tree stocking and little active management to retain live branches resulted in an abundance of small black knots in the timber and small tree diameters. However, it should be noted that small black knots are not undesirable for non-appearance framing products and planting at a high stocking for small knots followed by progressive thinning does produce good log grades and sufficient stem diameters. Active management could potentially take advantage of a high initial stocking to reduce branch size and pruning costs. Cost-effective alternatives to chainsaw thinning trees include ringbarking, which may be suitable for multiple progressive thinning operations in highly stocked stands (Satchell, 2018).
Root metrics and young trees
Phillips et al. (2015b) concluded that deficiencies in knowledge for below-ground traits hampers the ability to predict what effects different types of vegetation have on slope stability and that improved root information is required for an improved understanding of how and when individual species control erosion and how effective they are at different planting densities (Phillips et al. 2015b). However, Phillips et al. (2015a; 2015b) acknowledged that obtaining information on root morphology is time consuming and expensive, especially for older trees. Knowles (2006), on the other hand, simply used above-ground biomass to represent soil reinforcement capacity because below-ground biomass tends to vary between species. Trees with less root biomass tend to have greater tensile strength, whereby comparative soil holding ability should be similar for all species (Knowles, 2006). Poplar and Douglas fir are species that have less root mass but higher tensile strength, which results in similar levels of protection per tree provided above-ground biomass is similar. (Hocking, 2006a).
Root distribution is highly dependent on local environmental conditions (Schwarz et al. 2016) such as "soil physical conditions, particularly stoniness, site and soil drainage conditions, depth to water table, bedrock conditions and the strength and permeability of strata" (Phillips et al. 2015a). Tree growth is also dependent on soil fertility and climatic conditions.
Root spread, root depth and total root length are useful metrics for predicting comparative soil reinforcement performance by species (Stokes et al. 2009; Phillips et al. 2011, 2013a, 2014, 2015b). Marden et al. (2016) considered root size, biomass, lateral spread, depth, cross-sectional area and the strength of individual live, dead and decaying roots to be the relevant below-ground metrics useful for determining soil reinforcement by roots according to tree species.
Phillips et al. (2012) raised the issue that there is no "standard" for describing structural roots and defined structural roots as being those with a diameter greater than 1 mm, because this size class is dominant and abundant in young redwood and radiata trees. However, previous studies had mostly defined roots with a diameter of greater than 2 mm as "structural", thereby limiting comparisons but also creating a new and useful metric, "total structural root length" (Phillips et al. 2012). However, Marden et al. (2016) used greater than 2 mm diameter when measuring total root length for 1-4 year old radiata pine. Clearly there needs to be greater consistency between studies when comparing roots of different species, given species differences in tensile strength and below-ground biomass. For example, roots in young redwood are finer and more numerous than radiata, which has fewer, thicker roots at corresponding distances from the stem (M. Schwarz pers. comm. as cited in Phillips et al. 2012). Also, species with "deeper and more complex woody root networks impart better protection against shallow landsliding than shallow rooting systems, though in some cases dense networks of shallow woody roots can create a reinforced membrane of lateral-acting strength that may hold the underlying soil in place" (Sidle et al. 1985 as cited in Phillips et al. 2012).
Root systems create both lateral and vertical soil reinforcement (Phillips et al. 2015a). Different species have different methods for reinforcing the soil, for example radiata pine quickly develops vertical taproots and sinker roots (Marden et al. 2016) whereas redwood produces mostly lateral roots (Meason et al. 2012). Although roots were found to be more numerous and total root length significantly greater for young redwood trees compared to radiata, "the below-ground biomass for a given root collar diameter showed no statistical difference between the two species" (Phillips et al. 2012). Despite young redwood trees having greater total root length than radiata (Phillips et al. 2013, 2015a), root collar diameter appears to be the more useful metric for determining soil reinforcement.
Root collar diameter is useful for inferring root and below-ground biomass in young trees, as a proxy for DBH which is not a reliable measurement for size in young trees (Phillips et al. 2012). There is a significant relationship between root collar diameter and below ground biomass, meaning that below ground biomass and root biomass can be estimated by measuring root collar diameter independently of species (Phillips et al. 2012; Marden et al. 2016).
Root collar diameter increases exponentially in size in radiata pine over the first four years (Marden et al. 2016), suggesting that the window of vulnerability increasingly closes as trees grow. In the period between four and eight years of age, radiata produces significantly more vertical roots, both structural roots below the root collar but also sinker roots growing from lateral roots (Marden et al. 2016). Within that four to eight year growth period, radiata biomass increases by 75% and radial roots extend an additional 2 m from the stem (Marden et al. 2016).
Marden et al. (2016) found that "92% of the root mass of 4-year-old radiata was located within a 0.5 m radius of the root bole" with multiple vertical structural roots located directly below the root bole along with sinker roots that developed from lateral roots reaching the same depth as the vertical structural roots (Marden et al. 2016). Root depth increased from a mean maximum of 0.96 m in year one to 2.81 m after four years (Marden et al. 2016). However, by year four only 4% of total below-ground biomass occurred below 1 m depth (Marden et al. 2016). Where soils were deep and did not impede root development, vertical roots grew rapidly whereas thin soils and the presence of bedrock limited development of vertical roots and root biomass (Marden et al. 2016).
Comparative data was measured by Phillips et al. (2015b) from three year old trees for several species considered to have potential for controlling erosion. This study showed considerable differences in root systems between species at age three, suggesting greater potential for erosion control in some species. For example alder had the greatest overall root length and below ground biomass along with significantly deeper roots than the other species, with a rapid root spread with dense branching lateral roots and a high root density, suggesting it is a species very well suited to erosion control (Phillips et al. 2015a; 2015b). Blackwood had the greatest root spread and cypress had the greatest above ground biomass, following closely behind alder in greatest total root length (Phillips et al. 2015b). At age three, cypress had over three times the total root length of radiata pine (Phillips et al. 2015b). Beyond general observations, it is not yet known whether such differences in root system morphology are as important as root biomass, or whether root biomass or soil reinforcement can be reliably predicted from above ground biomass. Certainly early growth rates did vary considerably between species but "it is accepted that real differences between species are unlikely to emerge until the plants are considerably older – at least beyond 4 years from planting" (Phillips et al. 2015b).
Based on the wide range of root morphologies for young trees of different species, Phillips et al. (2015b) suggested that root reinforcement capacity could be inferred from measuring lateral root spread or total root length from three year old trees (Phillips et al. 2015b). Root measurements taken by Phillips et al. (2015b) showed that both redwood and radiata at age three "have greater than 50% of their total root length within 1m of the stem and show a rapid linear decline in total root length with radial distance" from stem, whereas blackwood and alder had less than 40% of the total root length within 1m of the stem with "a pattern of increasing total root length with distance from the stem to a maximum at about 1.5–2.0 m and then declining". Such metrics could potentially be used for modelling length of time required at a specific planting density for achieving 100% root site occupancy. However, exactly what metrics have the greatest influence over soil reinforcement is yet to be determined.
Disclaimer: The opinions and information provided in this report have been provided in good faith and on the basis that every endeavour has been made to be accurate and not misleading and to exercise reasonable care, skill and judgement in providing such opinions and information. The Author and NZFFA will not be responsible if information is inaccurate or not up to date, nor will we be responsible if you use or rely on the information in any way.
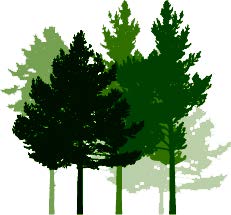